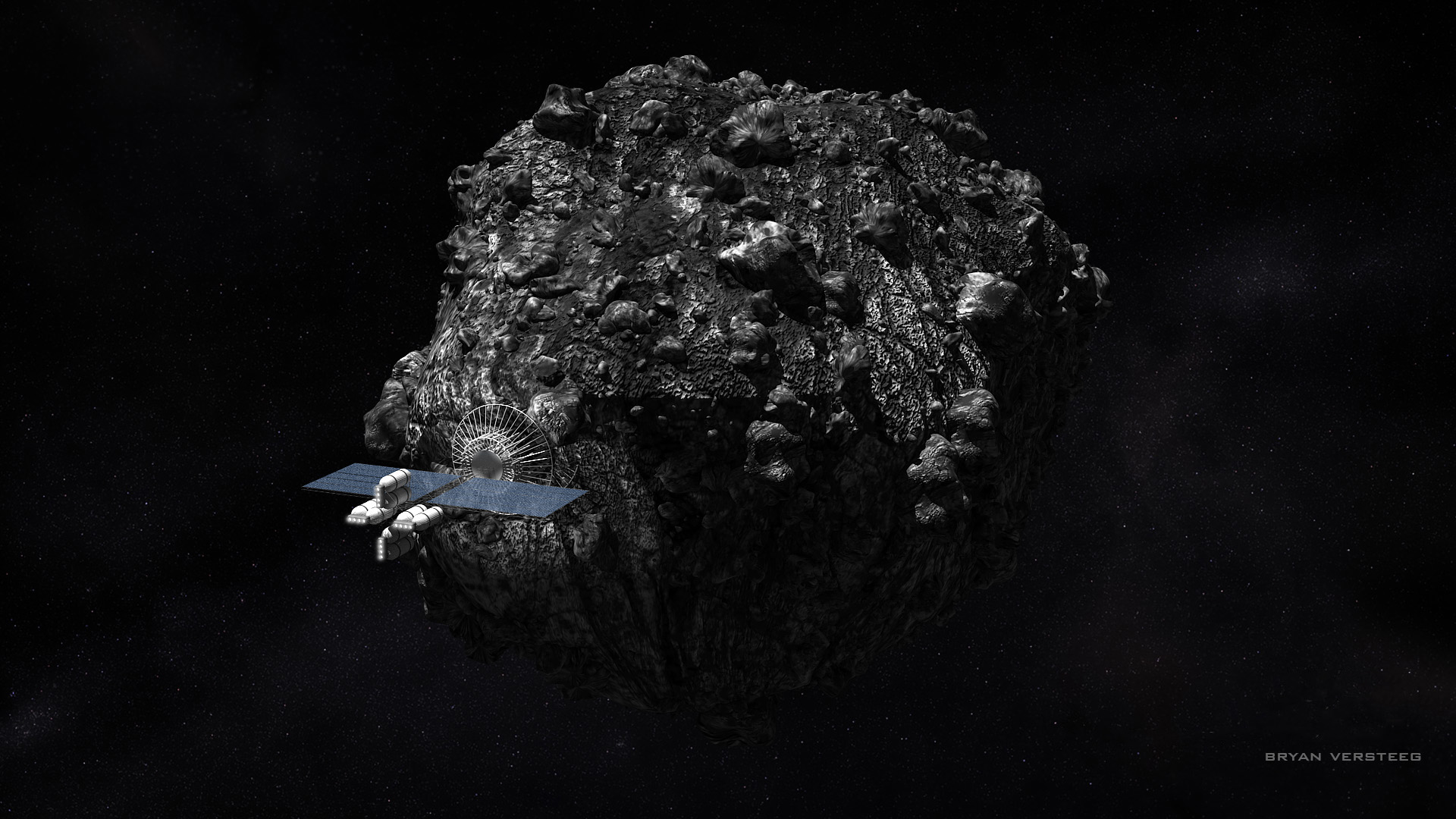
Asteroid ‘Target Body’ Models
Asteroid ‘Target Body’ Models
Introduction
In terrestrial mine project planning, it is important to develop an as-accurate-as-possible ‘orebody model’. This is needed for two purposes: (i) to plan the mining system (stoping system) so as to obtain best resource recovery; (and indeed the entire mine project depends on an adequate under standing of the orebody); and (ii) to enable a robust and supportable estimate of the total recoverable resource, for information to investors. Without an accurate orebody model neither of these two tasks can be accurately addressed. In hard rock mining, orebodies are generally well-defined volumes of ground which are many times higher in grade in the target metal than the surrounding country rock.The lateral and vertical extent of the orebody is bound by (generally but not always) fairly clear grade cutoffs, which may be depositional related or fault related, and the genesis of the orebody will explain the overall shape in terms of the original transport mechanisms which delivered the mineral values to the volume and deposited them there.
Whereas we elsewhere have discussed inferences regarding the mineralogical compositions of asteroids, this text considers inferences regarding the structures of our potential targets, both for the surface and near-surface regolith, and for the whole-body structure.
In this regard, we follow the conceptual framework of terrestrial geoscientists, where ‘orebody shape and genesis models’ are developed and tested against the various data sources which become available to them as a body of mineralization is investigated (remote imagery, remote sounding including seismic / radar, geophysical, and ultimately surface and drill hole sampling).
Analogously, for asteroidal resources we will need to develop target body models (which form the basis for making hypotheses about subsurface macro porosity and its potential to contain ice, for example) and ‘regolith development models’ (which might make assertions about surface layer grainsize and composition distributions profiles, and resultant cohesiveness and hence diggability, and amenability to selective beneficiation).
In the process of planning for docking onto and investigating our target asteroids, and excavating, handling, and processing asteroidal surface and subsurface material (regolith), it is necessary to explicitly recognize that we in fact do not know ‘what it’s like’. To systematize and control for that lack of knowledge, we need to hypothesize and explicitly define alternative ‘Target Body Models’ and hypothesize their engineering, thermal, and chemical properties.
These hypotheses (target body models and regolith models) regarding surface and at-depth structure, strength, and composition then guide the selection of anchoring, excavation, material shandling, and materials processing choices, in each case from a ‘portfolio’ of alternative technology options. Our miner and processor must be ‘fit for task’.
These various ‘body and surface type hypotheses’ will also guide the selection of our instrument choices, for initial prospecting (or more accurately, geotechnical test work) missions, as they highlight the most critical test data needed to drive the miner and processor design choices.
Asteroid Surface (Regolith Gener ation and Properties) Models: Discussion
In the 1990s, before the initial asteroid flybys performed during the Galileo mission enroute to Jupiter, consensus belief expected any asteroid less than several km in size would be a bare rock monolith, but all the close-in photos taken to date (Gaspra, Ida and its moon Dactyl, Mathilde, Eros, Lutetia, Itokawa, Toutatis, noting that only four are under 20 km size) show that all sizes of targets so far seen (down to 500 meters) are draped with regolith (dust, sand, pebbles, rocks). The same isseen with the fascinating images from comet 67P Churyumov-Gerasimenko: the surface is (variously) bare cliff and rock, gravel fields, and sand dune fields. This fits with flyby images of several other comets, imaged at much lower resolution.
It is now suspected that all bodies down to a much smaller size (100 meters or less?) retain a significant amount of their generated regolith. Since our target bodies must be of a size that can retain regolith, and given that the preferred minimum diameter cutoff is about 100 meters, due to need for visual acquisition prior to arrival, this is an important inference. Whereas regolith was previously believed (as for the Moon) to have been generated primarily by micrometeorite impact, and thus leaving ejecta fragments with significant kinetic energy1, it is now believed (Delbo et al) that the major regolith-generating mechanism is via thermal shock fracture, enabling retention of material at much smaller self-gravity. Loose regolith is positive for asteroid miners because it is easy to gather. Images of landslips on Eros and Gaspra show regolith on these larger bodies likely will be meters to tens of meters thick.
While the core of dark asteroids is fairly certain to contain volatiles, a major question is the water content of the top layers that are exposed to solar heating: How deep do we have to excavate to beassured of hitting water-bearing materials? Observations of 67P suggest significant variability in this depth, depending on the present or past mobility of the surface. Inferences from 67P will be most relevant for the presumed ‘comet-analog’ asteroid types, being the P and possibly D types.
How deep does the desiccation go? The answer would appear to be: less than on Earth. On Earth, agricultural studies show that the diurnal thermal wave penetrates 20 cm or so, depending on the soil grain-contact conductivity and (importantly) on its permeability to gas and vapor; and the annual thermal cycle penetrates to a meter or so. With the shorter diurnal periods (than 24 hours) of asteroids, larger surface temperature swings (+200C to-100C??), and in absence of significant gas transport of heat, we expect the temperature gradient to be very much steeper, and the desiccation layer to be significantly shallower. Models developed to describe comet surfaces infer adessication depth of a meter or so.
The average velocity differential between objects in the asteroid belt is about 5 km/s
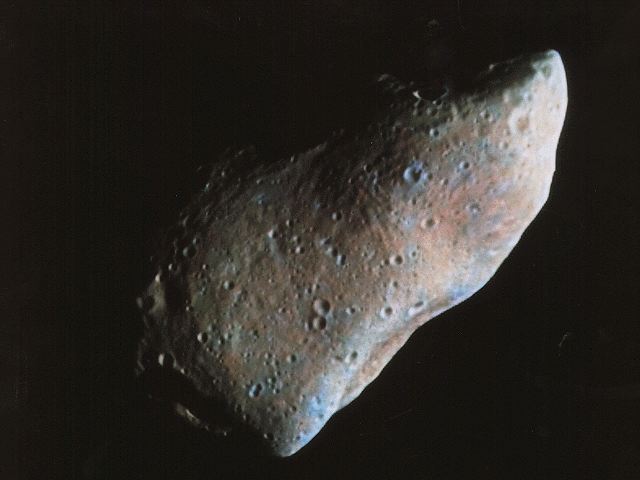
Fig 4.1 Gaspra, Main belt, S type
Gaspra (Fig. 4.1 above) shows a body shaped by flat ‘facets’, suggestive of an underlying monolith, but coated (draped) with a highly cratered (gardened) regolith.
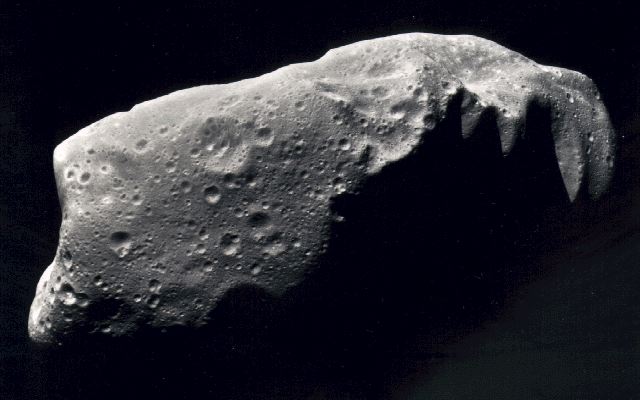
Figure 4 - 2 – Ida : main belt, silicate, apparently a monolith draped with regolith.
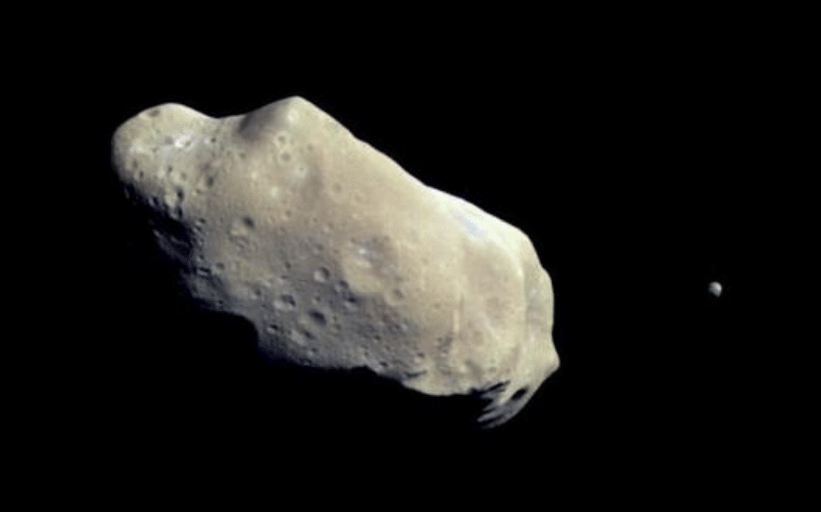
Figure 4 - 3 – Ida and its moon Dactyl
This image of Ida (above) provided the first unequivocal proof that asteroids can have moons. Again, there is evidence of significantly thick regolith (Jeffrey Kargel suggested ‘in excess of 100 meters depth’).
Galileo flyby images of Gaspra and Ida were the first unequivocal signs that asteroids can retain significant thicknesses of regolith, showing landslips and craters in thick regolith.
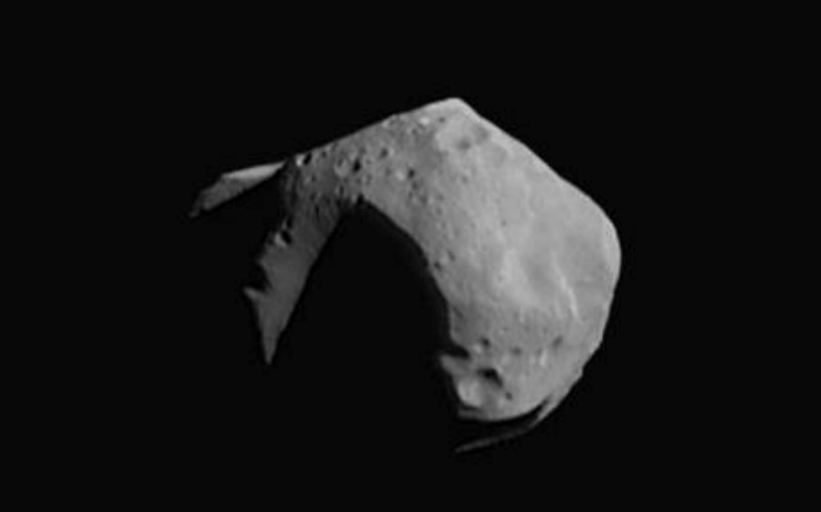
Figure 4 - 4 – Mathilde : Main Belt, C - class
More recently, close images were obtained from the NEAR probe of Mathilde, a Main-Belt C-class asteroid, in 1997 during its passage to its target, Eros, a NEA
Mathilde was noteworthy because the impacts which excavated the massive craters should have been big enough to catastrophically disrupt the body. The fact that it survives suggests (as does its very low density of 1.3 g/cc) that it is very highly porous (estimated at ~ 50%), thus dissipating the impact shock by collapse.
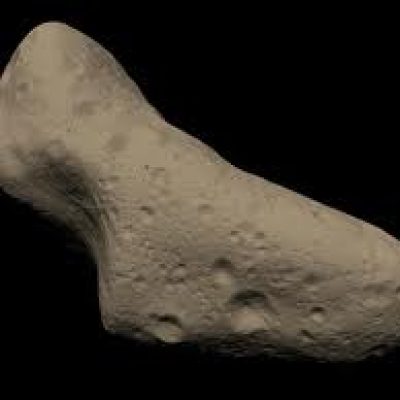
Figure 4 - 5 – Eros, NEA: S-class; monolith draped with regolith
Eros was the first NEA visited and orbited by a space probe, NEAR-Shoemaker, in February 2000
Itokawa, below, long axis dimension 540 meters, was visited by the Japanese Hayabusa probe in2005 which despite several failures succeeded in returning some grains of surface fines to Earth. Note the massive surface boulders, potentially from the “Brazil nut” effect where by seismic shaking, smaller objects gradually sink in a pile leaving larger ones at the top. Also note the ‘seas’ of smoother terrain, which is apparently of gravel or sand size. Itokawa’s density, measured to be 1.9t/m3, is very low, again indicative of high porosity.
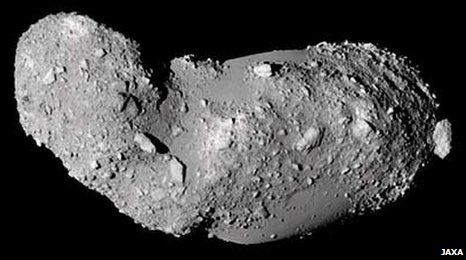
Figure 4 - 6 – Itokawa : NEA, S - type . Note massive surface boulders, potentially from the “Brazil nut” effect where smaller objects gradually sink in a pile leaving larger ones at the top.
Toutatis, below, was imaged by the Chinese Chang-e 2 re-purposed lunar probe in December 2013during its extremely close fast flyby. Again, photos reveal regolith, showing craters, and comprising both fines and big boulders, on a 4 km long body, which looks like a contact binary(two bodies in contact joined by a regolith and rubble neck).
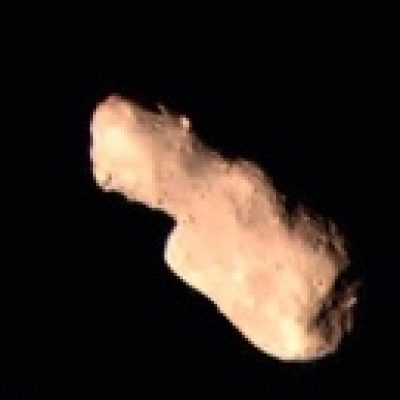
Descriptions of Various Possible Surface Models
Model 1 - ‘Gravel Pile’
In this model, we assume a very weakly cohesive or totally non-cohesive rubble pile or gravel pile, probably poorly sorted (i.e. having a very wide particle size range), similar to coarse ‘crusher-run’ unsorted gravel as used for road base. There may be a ‘Brazil Nut Effect’ present, whereby large particles are slowly surfacing, driven by seismic shaking. Also note that irregular surface properties and cracks will produce exaggerated differential weathering effects over time.
There is the probability (for all models) of impacts by meteorites causing shock lithification, which may have produced a hardened crust over time.
There may also be ongoing loss of surface fines, by ablation by micrometeorites, or by electro static charging and repulsion, such that sub-millimeter particles are being lost via levitation. Thus we might see coarser material on surface grading to finer material at depth. This might be analogous to ablation generated gravel pavement in the desert (gibber).
Observation: this means that any excavation method needs to be robust enough to penetrate a hardor resistant surface crust, gravel, or hardpan.
The images of Itokawa are generally thought to be very consistent with a Rubble Pile Model for the internal structure, with very coarse rubble gathered gravitationally around two massive clasts surviving from breakup of the original body, all draped with varying smaller sized rubble and gravel.
Sandy variant
Similar to above but with more fines on surface, draping as a fines layer over coarserrubble and massive boulders and (possibly) re-assembled 100-metre shards of the pre-impact body(‘megaregolith’) at depth. Some commentators believe this approximates to Itokawa. (This is in facta variant of Model 1.)
The Japanese probe Hayabusa touched down on (and sampled) Itokawa, a 500 meter long NEA, and its images showed ‘seas’ comprising gravel-sized regolith in gravity basins, and highlands of coarseand extremely large boulder fields. The bi-lobal shape of the body begs for interpretation as two re-assembled sub-bodies (‘mega-clasts’)
‘Higher strength’ variant:
Assume a more cohesive binding, mediated by electrostatic forces, by chemical bonding, by shock lithification, or more generally by Van der Waals forces, or by some form of vacuum welding, but with similar size ranges.
‘BullDust variant
Terrestrially, “bulldust” is a very fine dust that stays unconsolidated; it splashes when disturbed.
This one fits into the spacecraft and mission designer’s ‘take great care’ category: The surface could be a deep loose dust bed: dry quicksand; extremely unconsolidated. This would be like what some people thought would swallow the early Lunar Landers, and why the very first thing Neil Armstrong reported on was the texture and strength of the surface.
ProfJohnS. Lewis warns that there could be a lag deposit of loosely packed, fine dust mantling certain dark bodies ,especially on extinct or dormant comets that have experienced loss of interstitial ice as a result of solar heating of their surfaces.
The dust pools on Eros appear to be very fine-grained and loose material. Possibly ‘bulldust’. Note however there may be sufficient van der Waals stickiness in vacuum to make even fine dust slightly (or even strongly) cohesive. The fines deposits on comet 67P imaged by the Rosetta mission are similar.
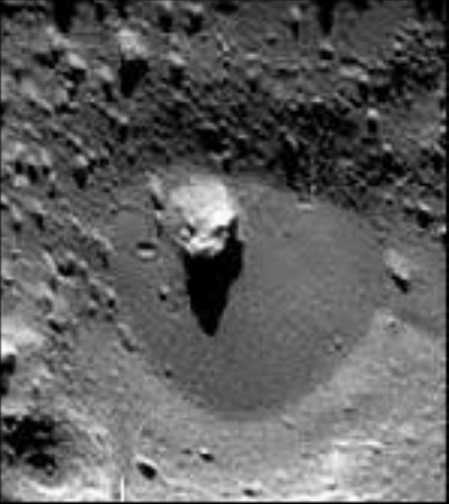
Initially the Eros dust pools were thought to result from electrostatic levitation and deposition, but a recent paper suggests fluidization by an impulsive release of subsurface gases.
Model 2 Cryptocomet:
Among the17,000+ discovered near Earth objects are at least few hundred dormant (or extinct?)comets, according to the interpretations of Shoemaker and others. Commentators believe that alarge proportion of the dark asteroids are in fact dormant or extinct comets. The ESA Rosetta mission to 67P Churyumov–Gerasimenko has provided a wealth of new information about what to expect when DSI begins resource extraction at objects which turn out to be dormant or extinct comets
Comets are basically porous icy dirtballs as seen on 67P Churyumov–Gerasimenko. Latest CONSERT data from Rosetta suggests whole-body porosity of 75 to 85%, and an ice to dirt ratio of 1:1 (with a factor of 2.5 either way!). Volume and mass measurements give a whole-body density of 0.45 t/m3.
So there is very significant porosity at depth, with a loose (or indurated / hard!) surface insulating layer that is devolatilized of free water but not necessarily of bound water. Both types of surface are clearly seen in 67P images.
Some models talk of the layer immediately under the insulation blanket as being densified by the downward mass transport of water vapor, refreezing to produce a hard permafrost
Thickness of layers of blanket (fluffy, sandy, scoria, gravelly lag deposit, or blacktop: totally unclear)and of hard permafrost might be each about 2 meters. (Recent interpretations of Rosetta images suggest loose dust layer thickness, where present, is a few cm to a few meters depth. Also notable is the large areas of 67P which are bare, apparently consolidated bedrock, whatever that is.)
Some aspects of this model may relate to observations on 67P Churyumov-Gerasimenko, including areas of apparent hardened surface, and the presence of apparently deep dust dunes, with Aeolian flow features, including wake deposits behind boulders; indicating the presence of very fine micronsized dust particles, and the suggestive presence of densified layering.
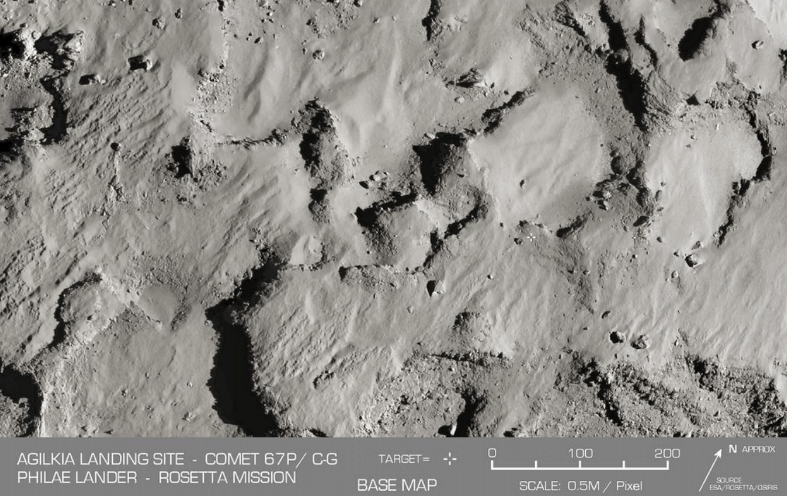
Figure 4 - 12 – Photos of Comet 67P show dust aeolian flow features .
Some of the apparent sandy / dusty layer (below) shows apparent fumarole-like features. The dust layer is apparently of some few meters thickness at most, as we can see from the out cropping bed rock.
The presence of boulders on the surface gives comfort that it has some bearing strength.
Figures 4-12 to 4-14 also show distinct indicators of strength variations: layers of varying resistance, ‘bedrock’, boulders, sand dunes, furrows, apparent fumaroles, etc.
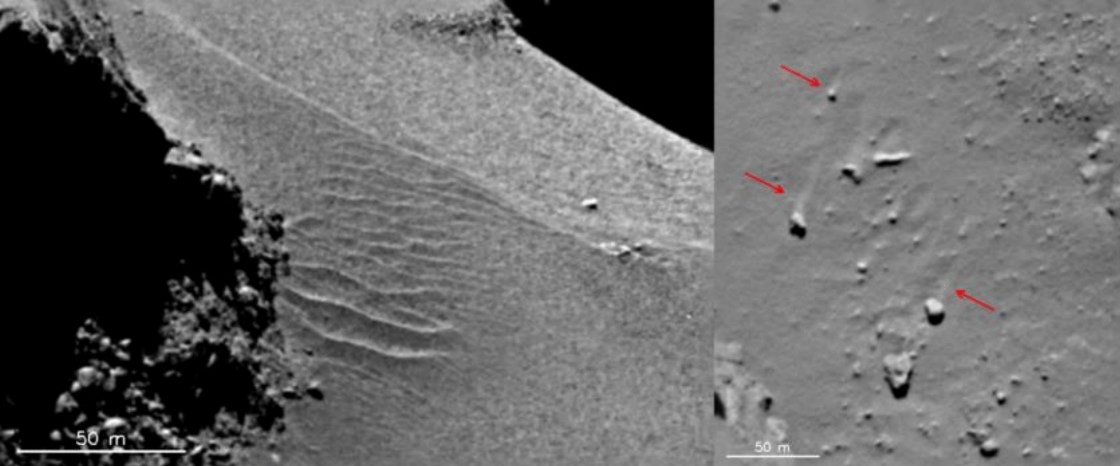
Figur e 4 - 13 – Proof of dust dunes and flow features ; tiny particles will be the easiest to process .
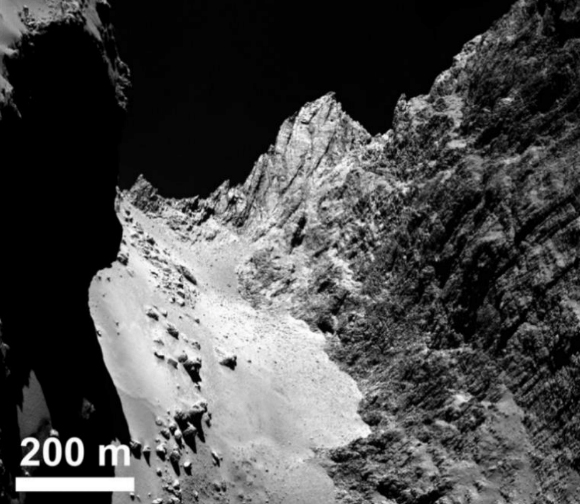
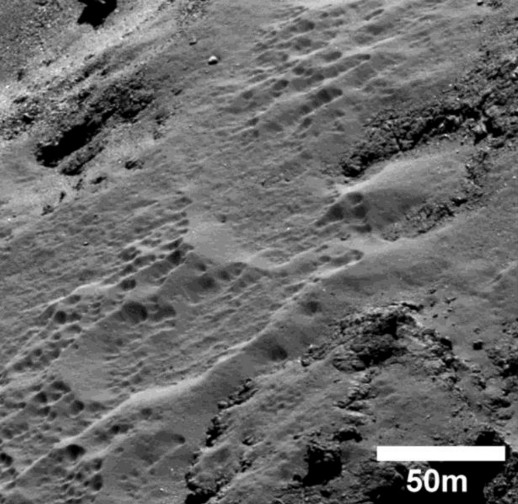
Figure 4 - 14 – Other parts of 67P seem to show layering, exhibited as lineaments in cliffs, and outcropping layers or emergent resistant ridges of apparently hard material. Right hand image shows fumarole, indi cating outgassing from a depth of some meters.
In Figure 4-16, a circular vertical-sided depression was photographed: this has been hypothesized tobe a collapse depression due to evisceration by sublimation of an underlying ice pocket (a bit like adoline in desert country generated by underlying limestone pockets that get leached away, allowing subsequent surface subsidence).
The fact that the Philae lander bounced (twice!) confirms however that at least some areas of the surface of this comet were hard, kinetically elastic, i.e. mechanically competent material.
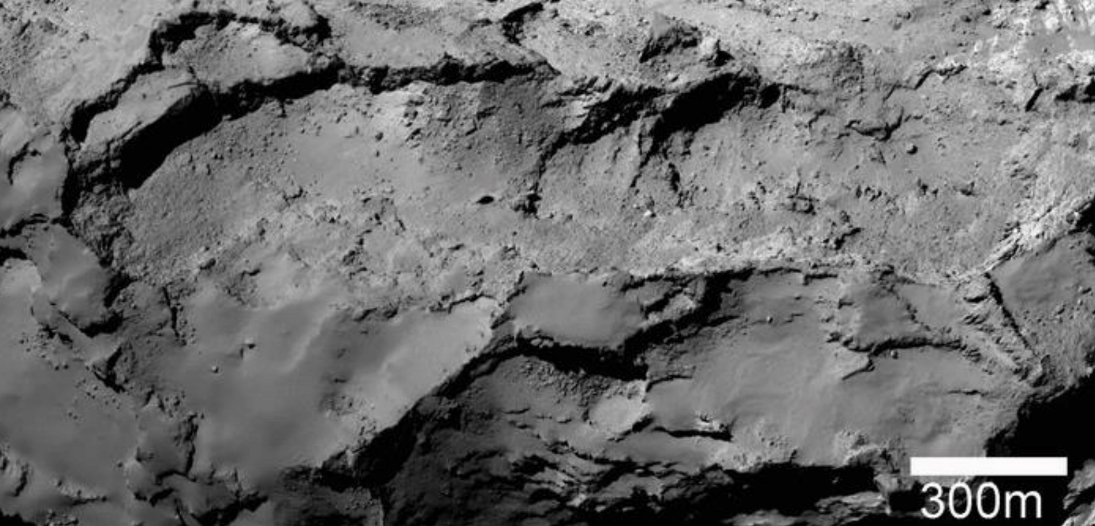
Figure 4 - 15 – This image seems to show resistant ridges or layers of apparently hard materials.
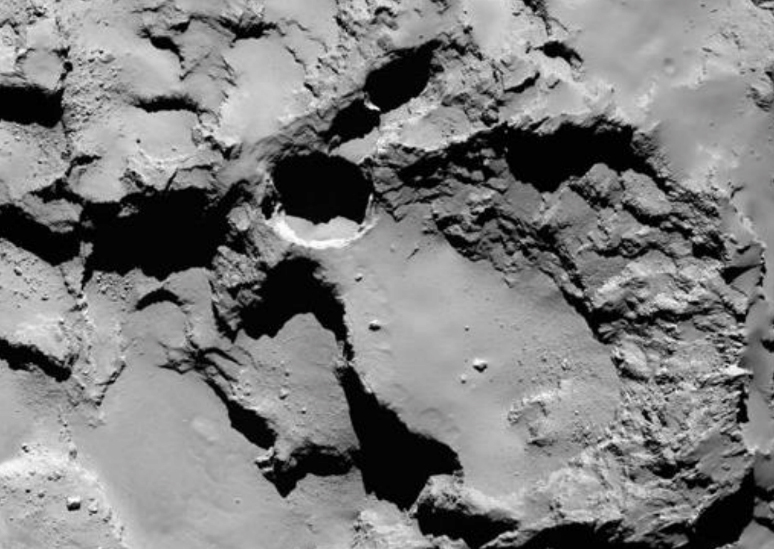
Figure 4 - 16 – A circular depression is visible in this image of Comet 67P.
A notable thought is that very loose and apparently fluffy but possibly surface-reactive fines can bond over time to a quite competent rock. This is a well-known occurrence with freshly released and thus surface-chemically-reactive mineral concentrates that are then stored for extended periods
Close-up images taken during the descent of the Philae lander show an extraordinary regolith comprising coarse clastic fragments (of several cm to tens of cm size) in a bed of finer material of grain size a few mm. This looks much like a scree slope.
The above array of surface features found on 67P can also be expected on D-type asteroids, which are a spectral match to the Tagish Lake meteorite, and also on P-type asteroids. These images thusare of great significance for designing the miner craft .
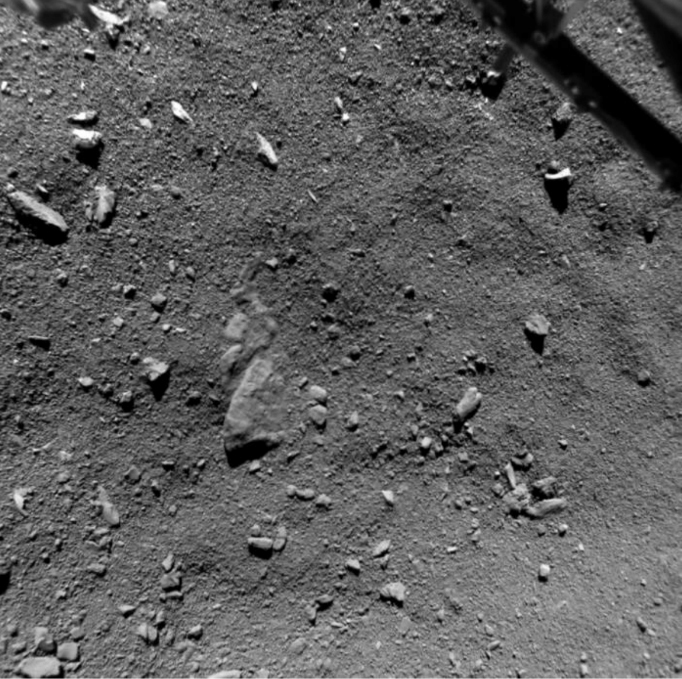
Figure 4 - 19 – Surface of 67P from nine meters altitude.
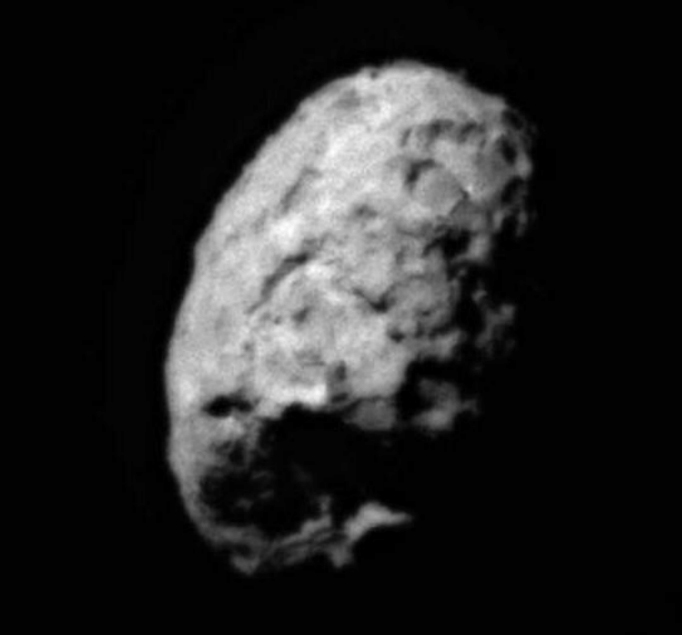
Figure 4 - 20 – Comet 81P/ Wild - 2 – 5.5 x 4 x 3.3 km ; note depressions
More flat-bottomed depressions are seen, albeit at lower resolution, here on Comet Wild-2(Stardust Mission). Comet density is 0.6 g/cc, so porous that a new understanding of “bedrock” is needed for the cometary context. Below is a stereo view from ‘the other end’.
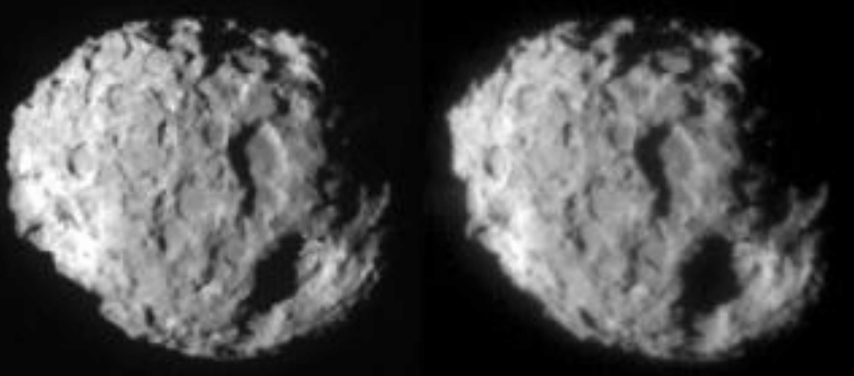
Figure 4 - 21 – Comet Wild - 2 in stereo : cross your eyes & focus on middle image to experience a 3 - D effect
Thus we seem to be seeing craters in several comets that have a ‘different morphology’ from that expected for impact origin: circular depressions with notably flat interior floors. CometWild-2 above shows them, and we see them in 67P Churyumov-Gerasimenko.
There is a suggestion these are collapse depressions above subsurface caverns which have hollowed out due to sublimation. (equivalent to the dolines in desert limestone terrain previously mentioned, or collapse depressions such as have recently been reported in Siberia, thought to be collapse caverns where subsurface clathrates have volatilized.
Comet 103P / Hartley-2 displays several questions about comets to which we don’t have answers at the moment: 1) what are the bright spots? Are they ice? And 2) why the different texture to the ‘neck’ (seen also in 67P)?
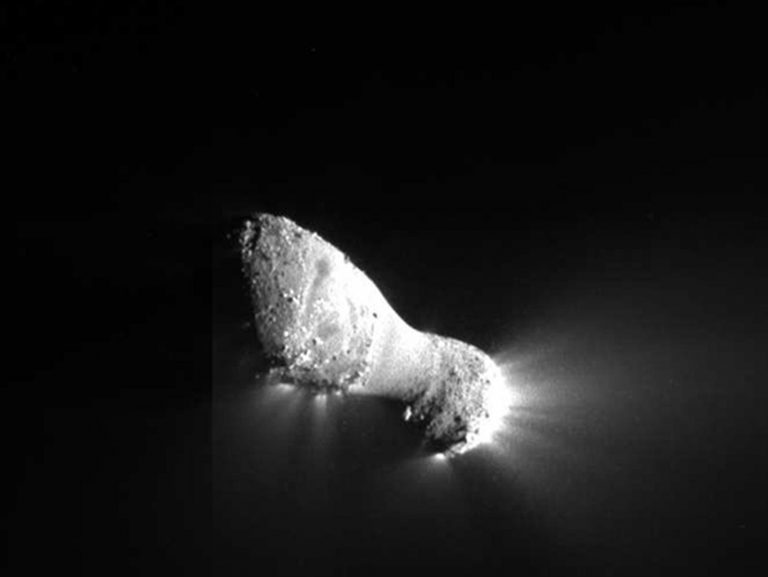